The seismic signature of a sedimentary rock is strongly influenced by the geological history of the rock, e.g., the depositional environment, whether it has experienced tectonic burial and uplift, etc. These processes have direct impact on mineralogy, degree of sorting, amount of compaction, amount of cementation, degree of chemical alterations, forming of fractures, etc., and is what we term the “memory of the rock”. This means that the same stratigraphic unit at two different locations can have a very different seismic amplitude response if the burial history changes between these two locations. Ignoring this fact can lead to inaccurate, misleading, and sometimes wrong conclusions from the seismic amplitude data analysis. Here we outline a workflow for consistent integration of the burial and diagenetic history of a sedimentary rock into quantitative seismic data analysis through consistent use of rock physics models.
Burial history of a clastic sedimentary rock
We can divide the burial history of a sedimentary rock into the following domains:
- Deposition / near surface domain
- Mechanic compaction domain
- Chemical compaction domain
- Deeply buried and subsequently uplifted domain
A seismic response is not caused by contrasts in the geological properties, but by the contrasts in the seismic properties associated with the geological properties, e.g., contrasts in P- and S-wave velocities and density. Therefore, the workflow needs to describe the impact each of these domains will have on the seismic properties of the rock.
Different lithologies, such as sandstones and shales, have different responses to the different domains. Subsidence of sandstones will cause a gradual stiffening of the rock texture primarily due to sorting, packing, and crushing of the mineral grains. This will reduce the porosity and gradually increase the seismic velocities and density.
A more dramatic change in elastic properties occurs when the quartz-rich sandstone enters the chemical compaction domain around 70°C, i.e., it is heated enough for quartz cementation to start. Even a small amount of cement at grain contacts is known to drastically stiffen the rock, and consequently increase the seismic velocities. The amount of cement is a function of the time spent in the chemical compaction domain; both during burial and uplift.
Finally, subsequent tectonic uplift with possibility for erosion and stress release may cause fractures and cracks in the cemented sandstone creating increased variability in rock texture and pore shapes.
On the other hand, shales are typically deposited as randomly oriented clay platelets with high porosity. This is an unstable structure that quickly collapses during mechanical compaction, with drastically reduced porosity and increased seismic velocities and density as consequences. Alignment of the platelets will also make the seismic velocities dependent on the angle of the seismic wave relative to the alignment of the platelets, i.e., anisotropic velocities.
Chemical compactions for shales occur at higher temperatures than for sandstones, typically in the interval 80-140°C, and are characterized by mineralogical transformations. One example is how smectite is altered to illite causing an increase in the seismic velocities.
The rock physics link between burial history and seismic properties
The physical link between burial history and seismic response is described by a rock physics model. For a rock physics model to be credible, it needs to capture not only the present-day geological characteristics of the rock, but also needs to be consistent with the “memory of the rock”. In addition, the rock physics model must remain consistent with the underlying physics of seismic wave propagation in complex heterogeneous media. This means that there is not a single rock physics model to be used throughout the burial history of a sedimentary rock, but rather a group of models to be used in each domain of the burial history.
An integrated modelling workflow has been developed to mimic how rock properties and associated seismic signatures change with the burial history. It can be used to investigate the feasibility to distinguish between different lithologies and pore fluids using seismic amplitude data. The main steps of the workflow are outlined in Figure 1.
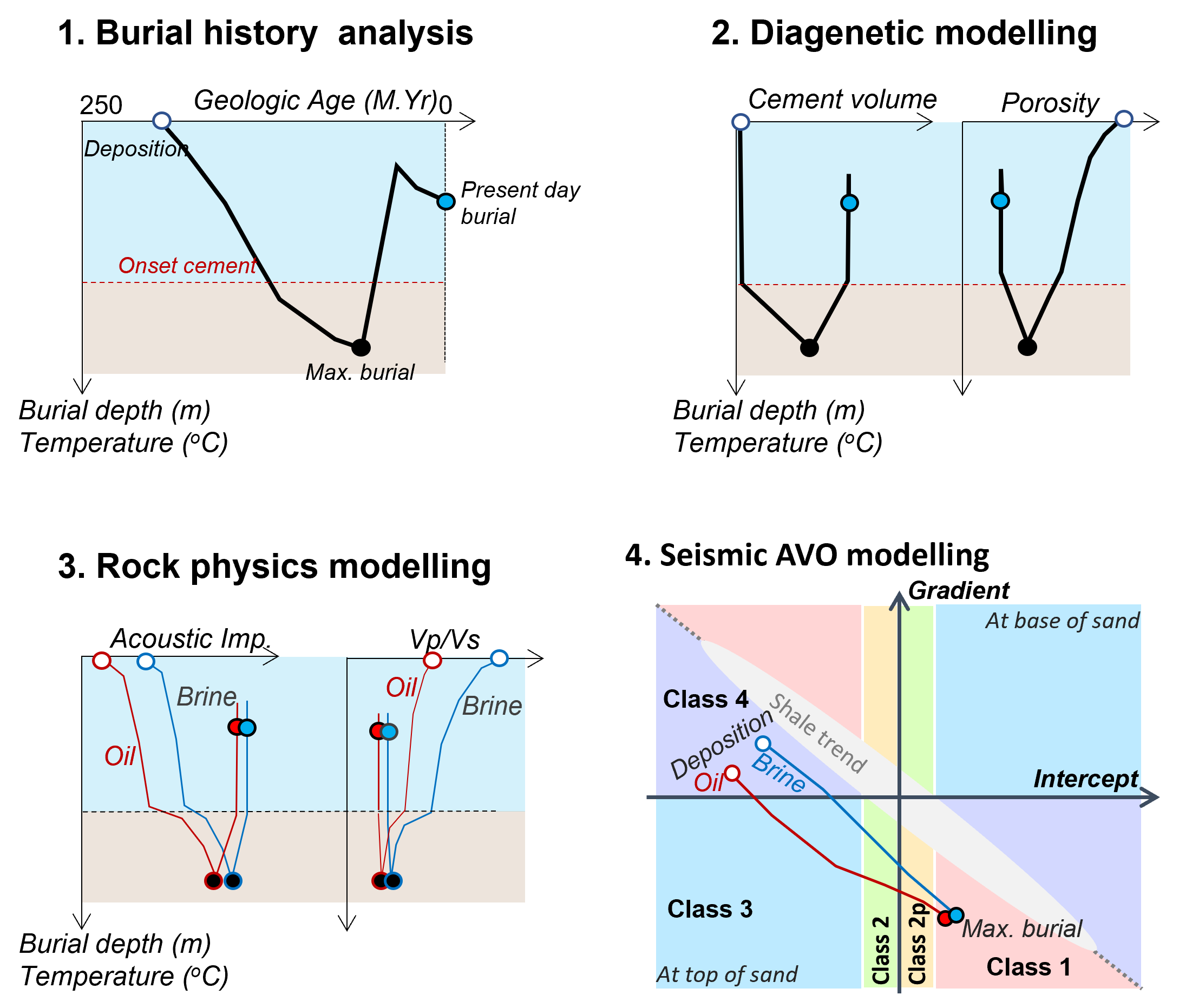
The workflow starts with burial history analysis at the key wells, constrained by basin modelling, seismic interval velocity data, and petrophysical and geochemical data. Uplift and associated net erosion are estimated from seismic interval velocities calibrated to well log observations. This enables us to predict porosity and cement volume as a function of geological age. These parameters are subsequently put into the appropriate rock physics model to predict the seismic property curves that correspond to the burial history curve. Finally, the seismic AVO signature is predicted by seismic modelling and can be displayed as intercept-gradient data. Seismic signatures are often shown as AVO classes defined from the intercept-gradient cross-plot, being an efficient way of scanning for seismic anomalies of geological interest.
An interactive tool has been developed specifically to calibrate the workflow parameters at well locations, and to validate the predicted burial history curve and associated seismic parameters and signatures. This also allows us to vary the burial history and automatically see the response in the diagenesis, the elastic rock properties, and the seismic signature. Performing sensitivity tests are important for evaluating the uncertainties of the workflow.
Having validated the input parameters, the workflow can be run in the whole area of interest. The results from are here presented as maps for a target surface (Figure 2). Note how the temperatures at maximum burial depth vary, implying that the degree of chemical compaction and quartz cementation will vary accordingly. In some areas, the target surface has not reached temperatures high enough to set off chemical compaction, and we should then expect unconsolidated sands.
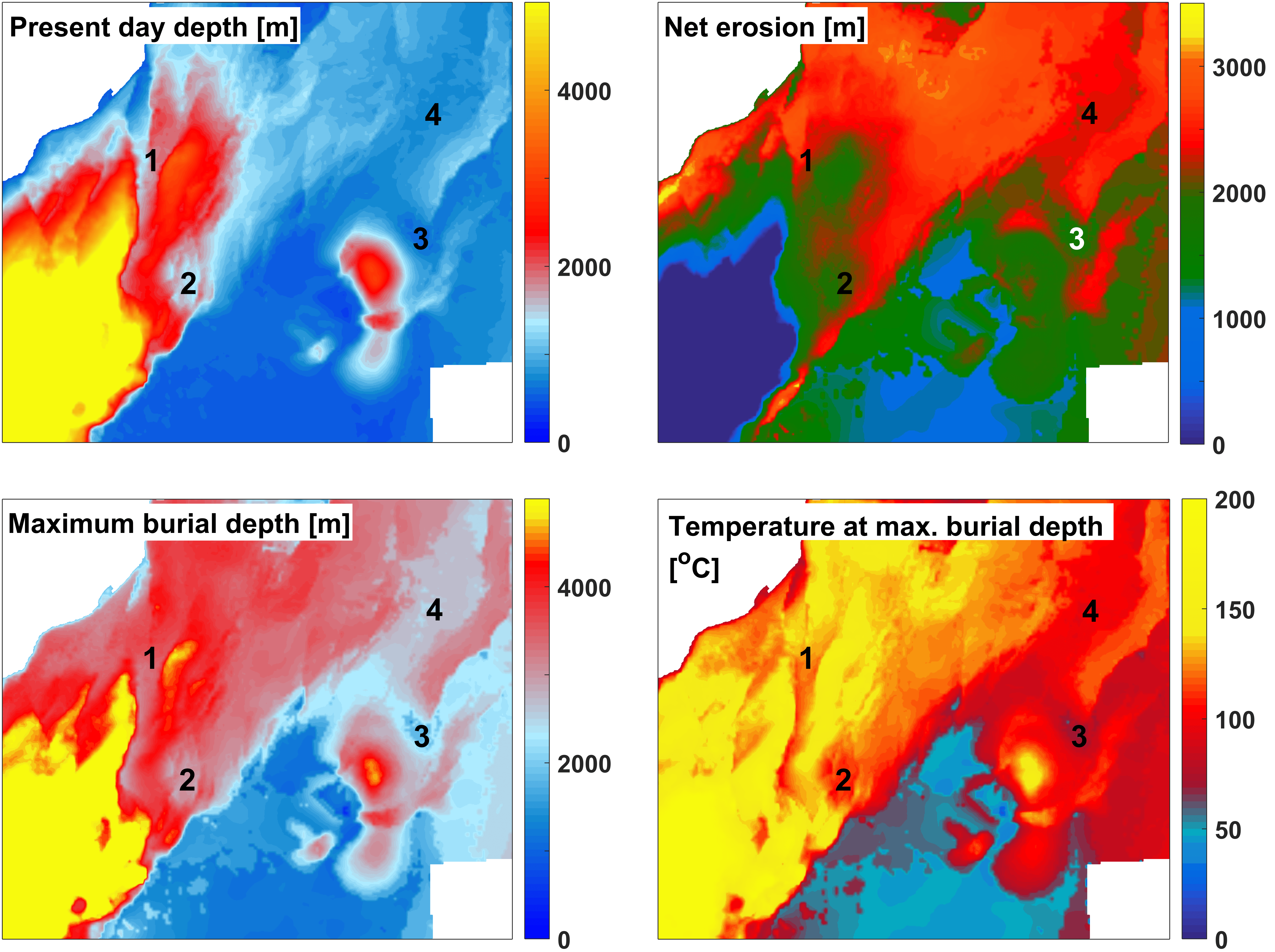
The final step of the workflow is to predict the seismic signatures for the target formation assuming different pore fluids to be present (Figure 3). In prospect 1 we find AVO class 1 for both oil and brine saturated sandstones. It will therefore not be possible to discriminate between the pore fluids at this location, probably due to the overlap in the elastic properties of the stiff cemented sandstone. However, in prospect 3 the seismic signature can still be quite different between brine and oil even though they belong to the same AVO class. In prospects 2 and 4, the seismic signature falls in different AVO classes depending on the pore fluid, indicating that there is high likelihood of separating between a brine- and an oil-filled sandstone at these locations using seismic amplitude data.
The AVO feasibility workflow can easily be run with many scenarios to evaluate the stability and the sensitivity of the predicted properties to uncertainties in the burial history analysis and the geological features being used in the analysis.
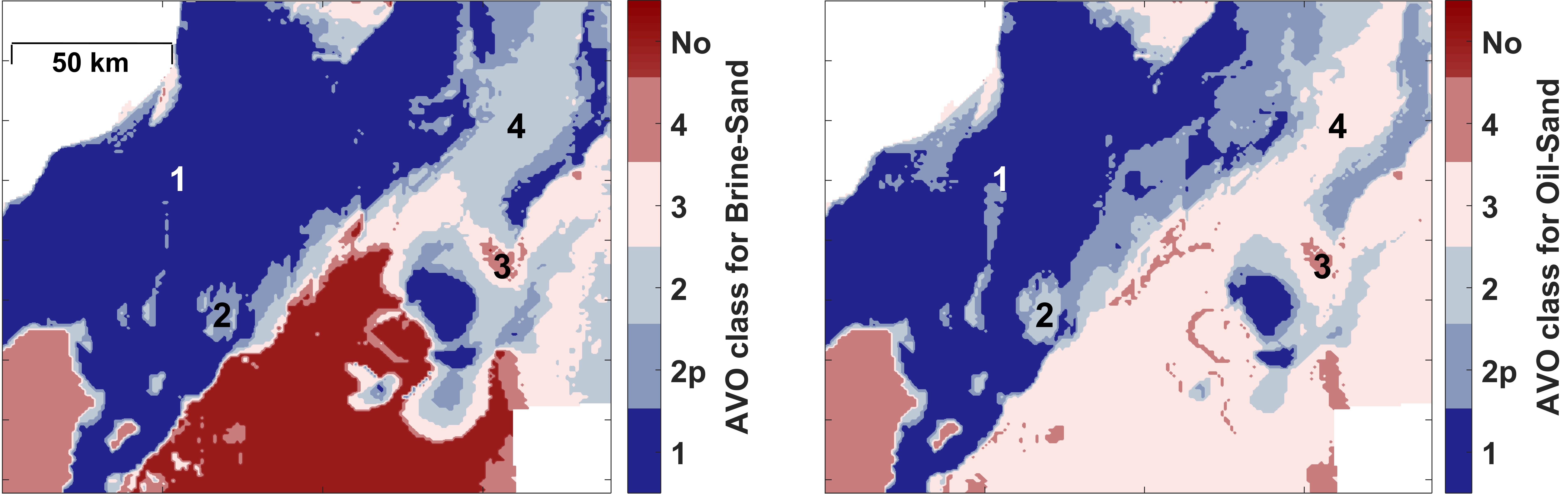
Drawing more accurate conclusions
Future subsurface exploration and maturation will likely need to focus on increasingly more subtle traps with subtle seismic signatures, e.g., located down-flank or up-dip from drilled/explored structures. Knowing which seismic amplitude signature that are associated with the different lithologies and fluids is essential for doing reliable geological interpretation of seismic amplitude data.
We have described a workflow that reduces the risk of drawing inaccurate or erroneous conclusions from seismic amplitude data, by considering the burial history of the formations of interest and integrating it with diagenetic, rock physics and seismic modelling.
References
Avseth, P., Mukerji, T., and Mavko, G. (2005), Quantitative seismic interpretation: Applying rock physics tools to reduce interpretation risk, Cambridge University Press.
Avseth, P., and Lehocki, I. (2016). Combining burial history and rock-physics modeling to constrain AVO analysis during exploration. The Leading Edge, 35, 528–534.
Avseth, P., Lehocki, I., Hansen, T., Angard, K., Schjeldrup, S., and Shelavina, E. (2020). “A new integrated workflow to generate AVO feasibility maps for prospect de-risking,” in Proceedings of the 82nd EAGE Annual Conference & Exhibition.
Lehocki, I., and Avseth, P. (2021). From cradle to grave: how burial history controls the rock-physics properties of quartzose sandstones. Geophysical Prospecting, 69, 629–649.